Analysis of in-vessel accident progression in VVER1000 NPP during SBO accident with external reactor vessel cooling method
In this study, the MELCOR v1.8.6 code was utilized to perform an analysis of the in-vessel
accident progression in VVER1000 reactor during the Station Black-Out (SBO) accident with and
without external reactor vessel cooling (ERVC) strategy. The analysis presented the predictions of the
main phenomena during the accident such as failure of fuel cladding, collapse of lower core support
plate, relocation of core debris to lower plenum and mass of debris components in lower plenum, and
provided comparisons between two cases in term of main parameters such as integrity time of reactor
and structure components of molten pool. These parameters are very important inputs for further
research on the application of external vessel cooling strategy for VVER1000 reactor.
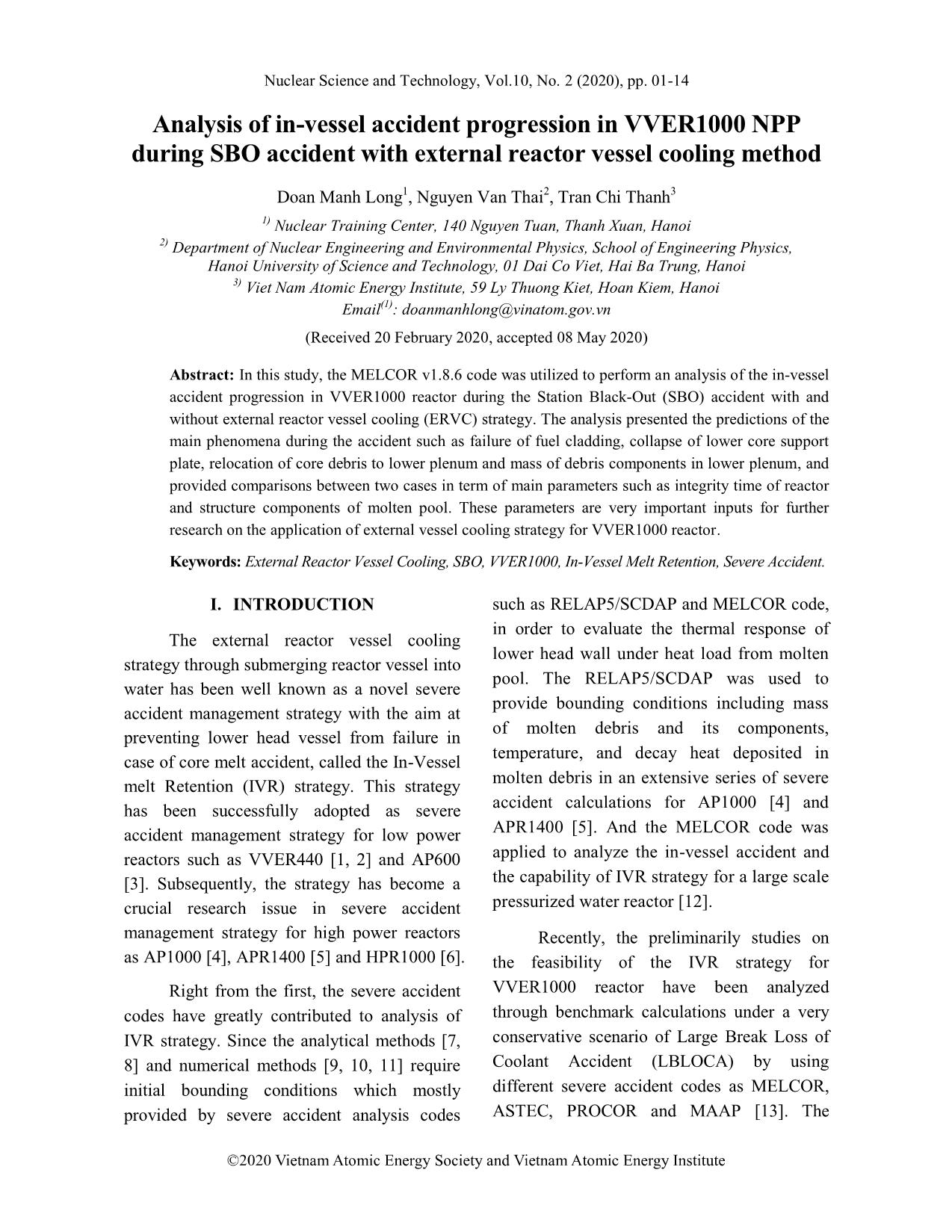
Trang 1
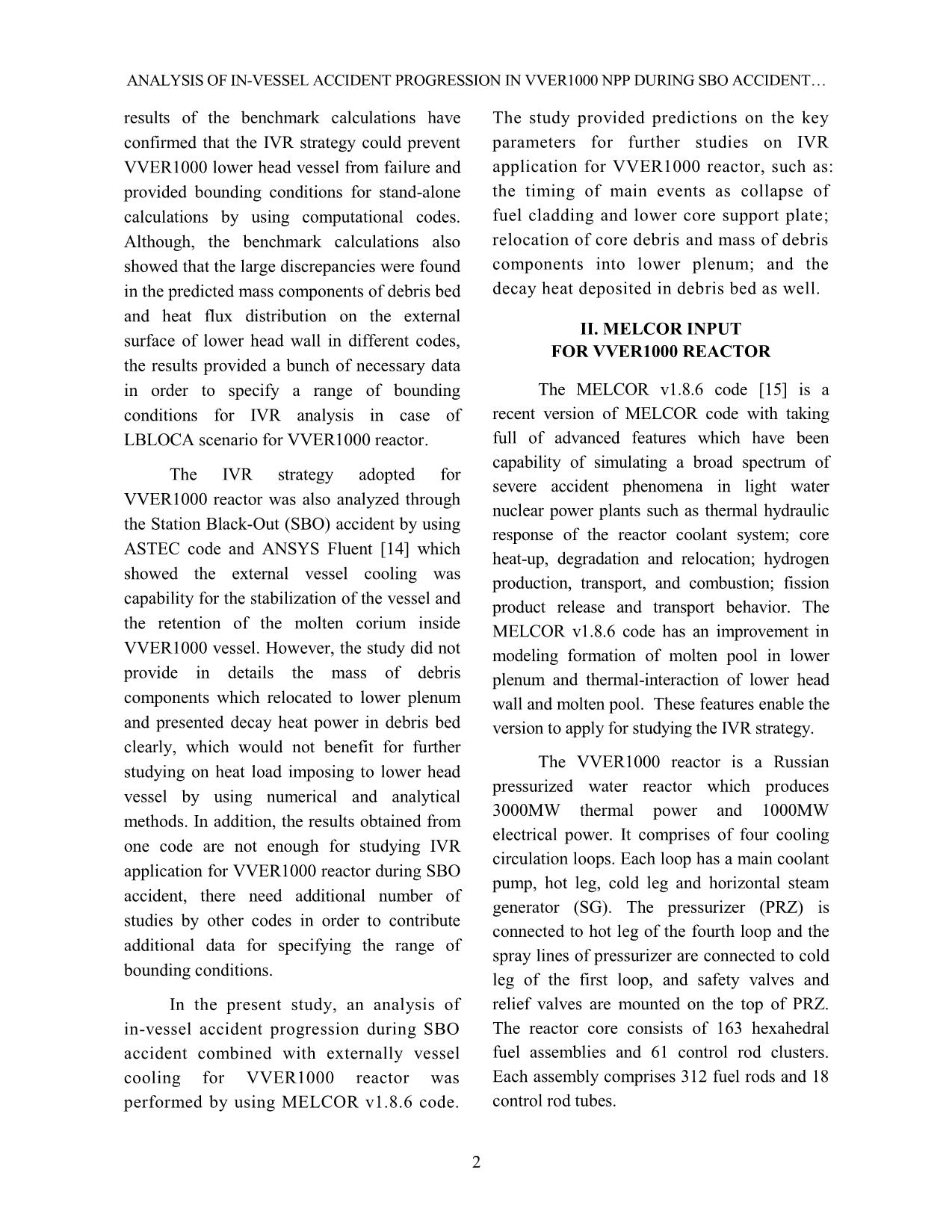
Trang 2
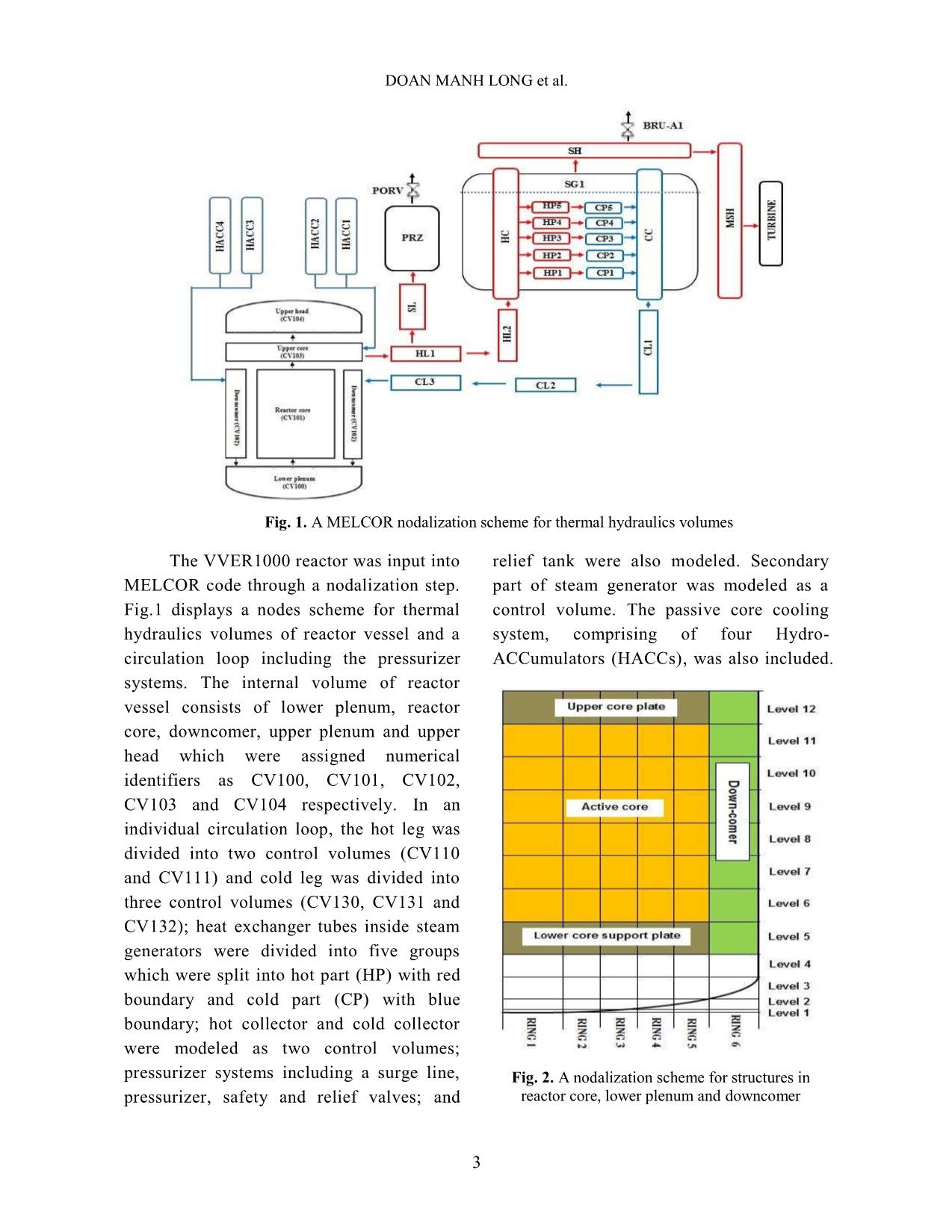
Trang 3
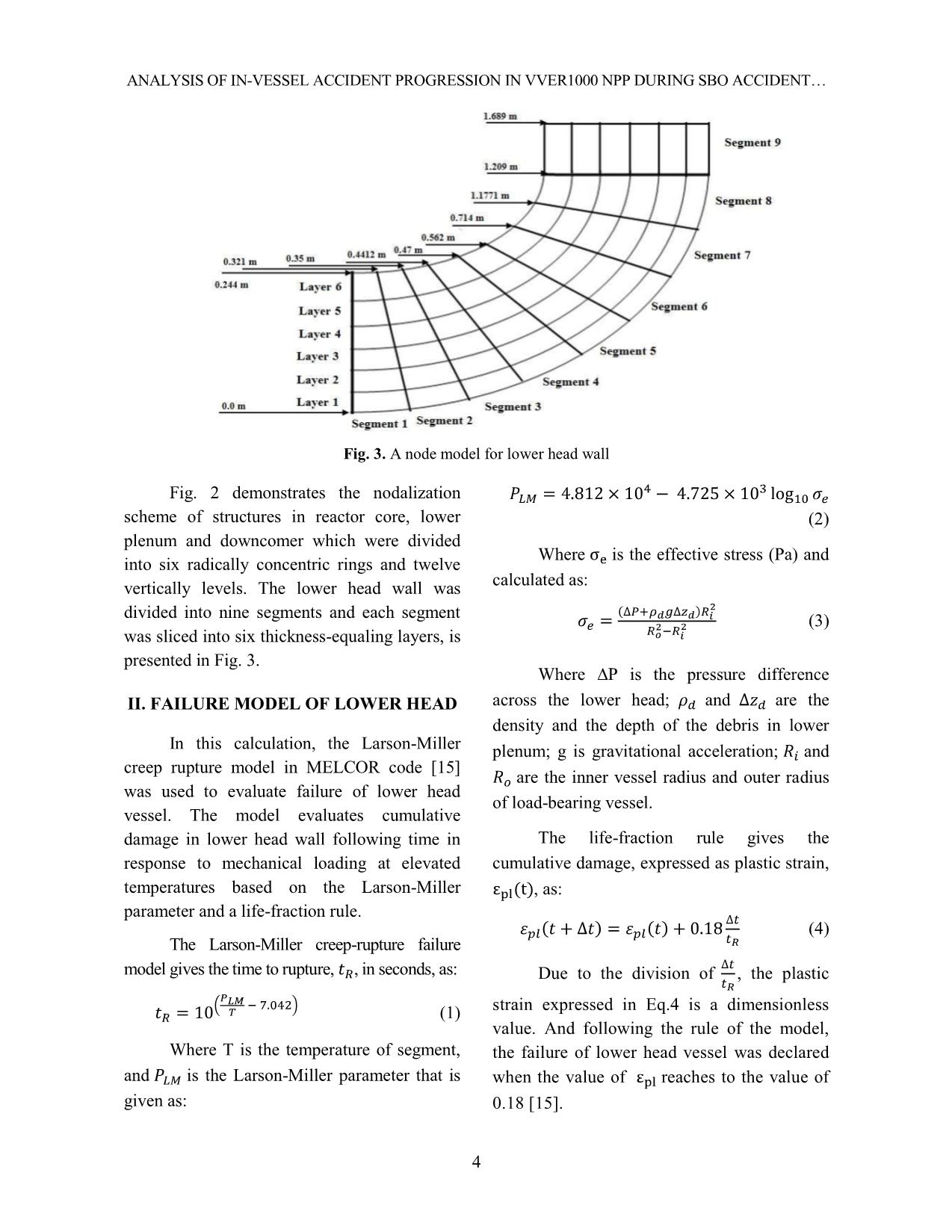
Trang 4
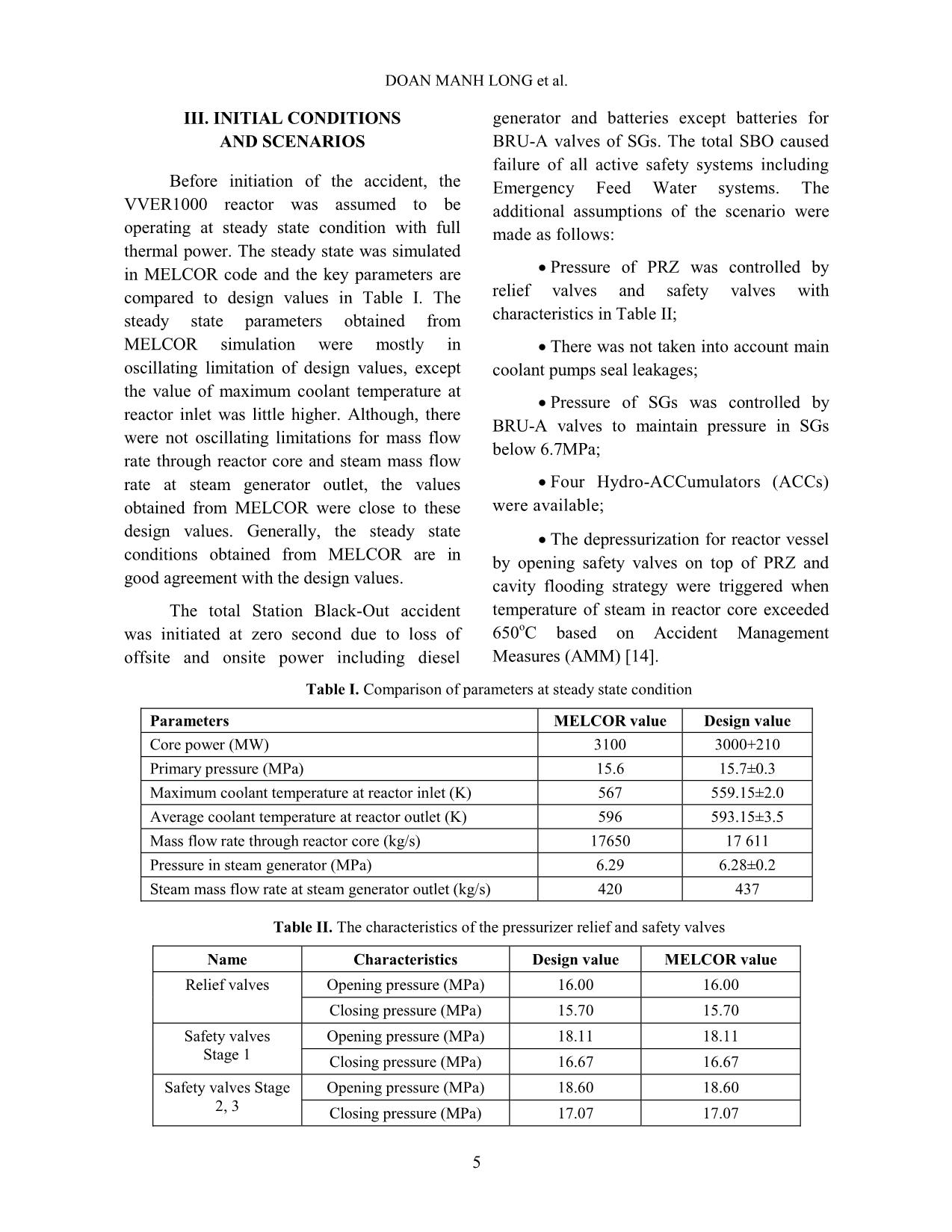
Trang 5
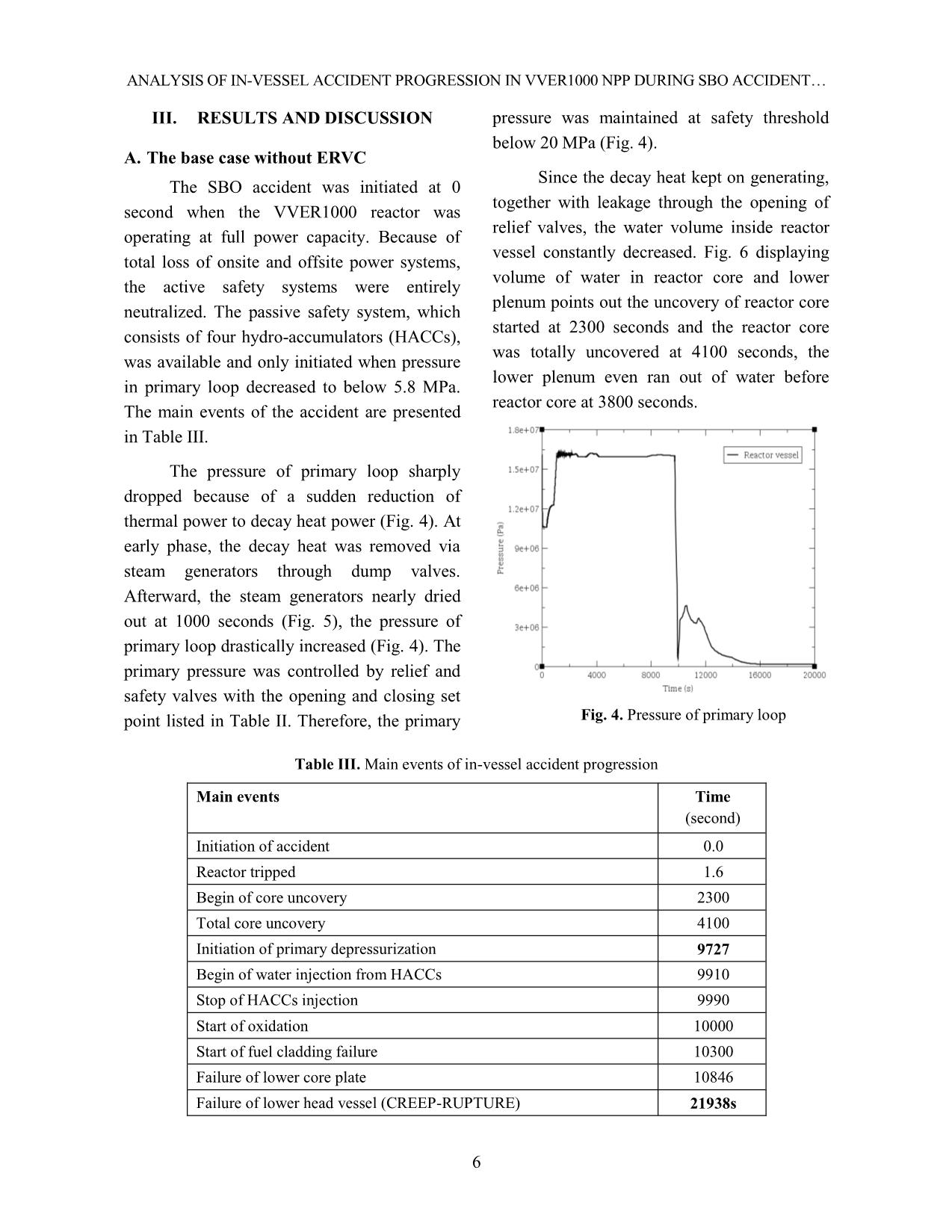
Trang 6
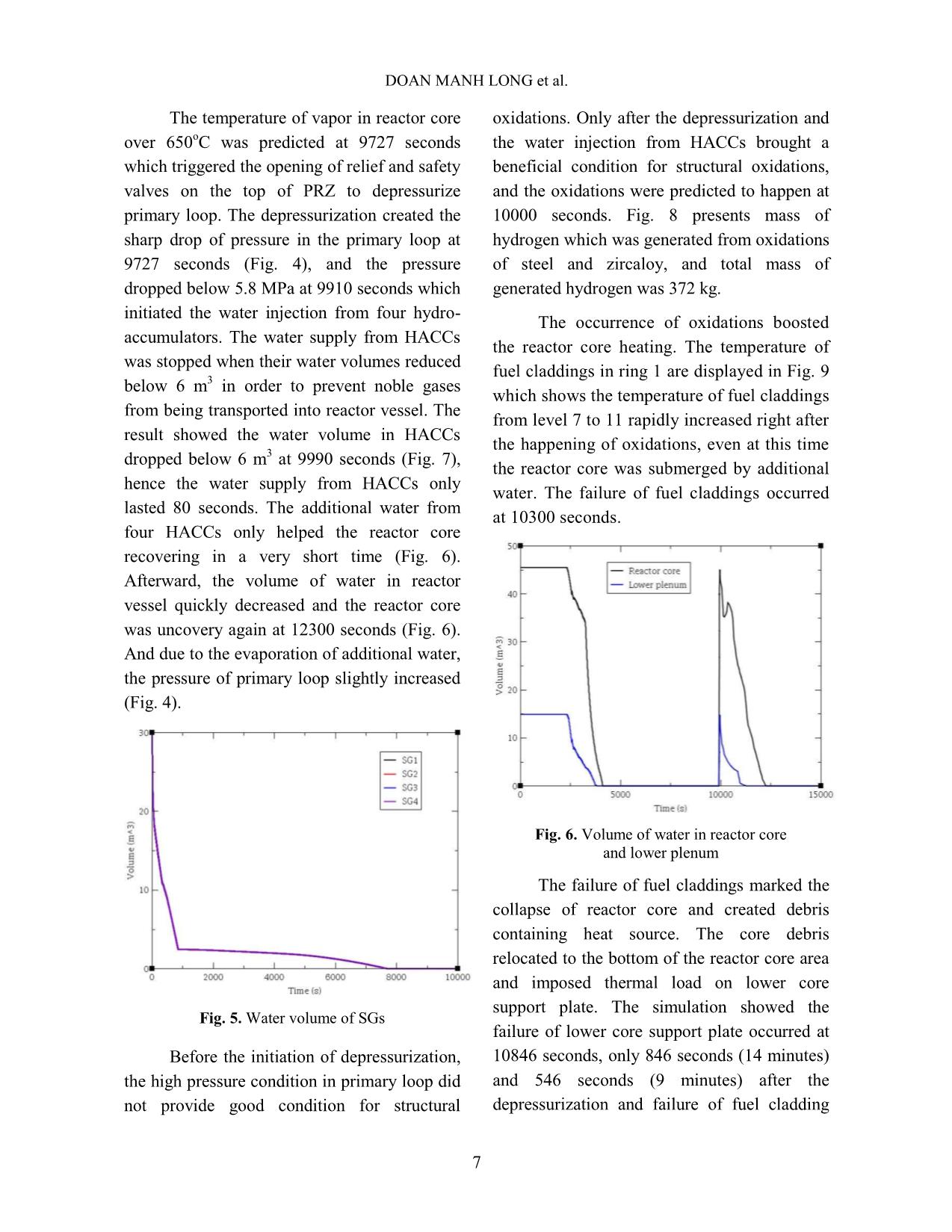
Trang 7
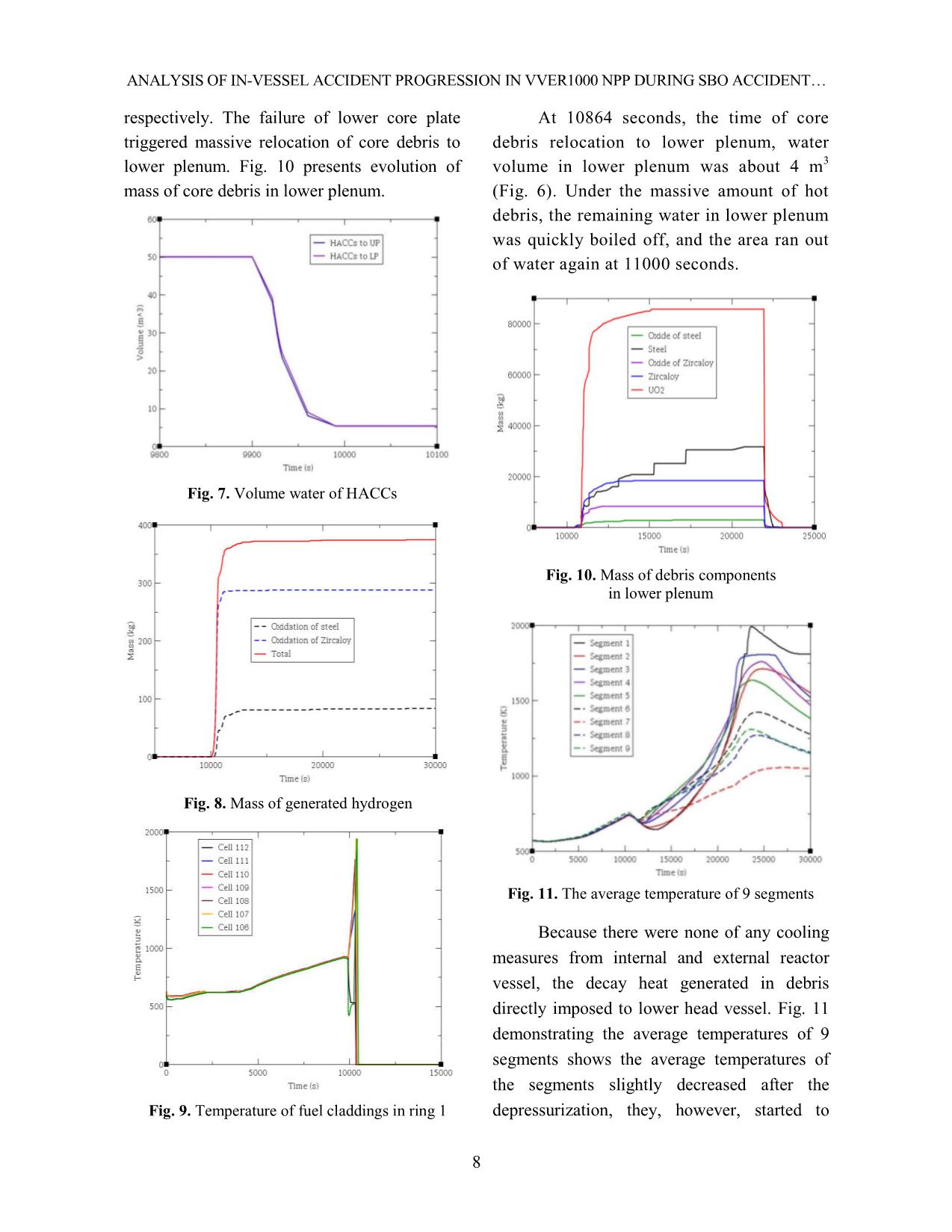
Trang 8
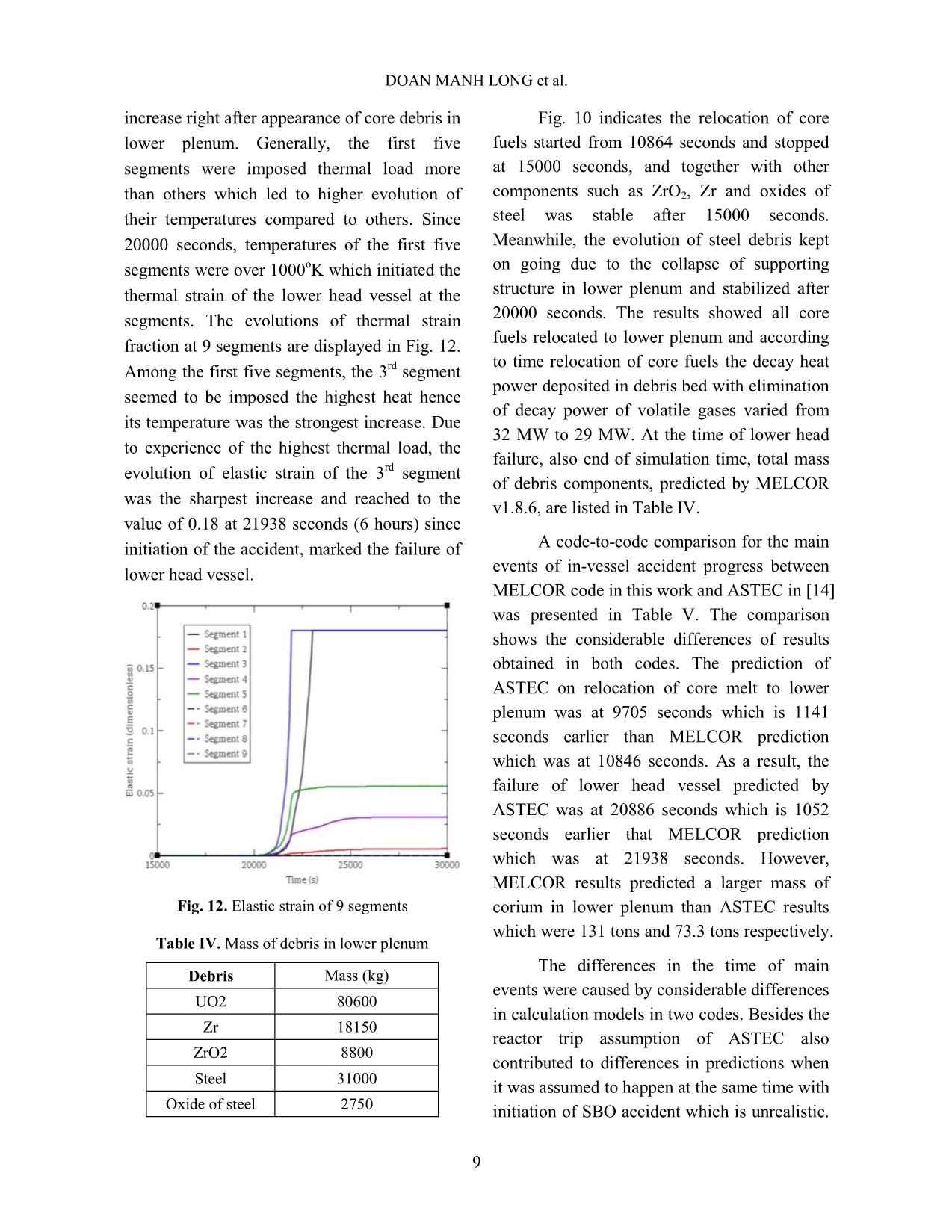
Trang 9
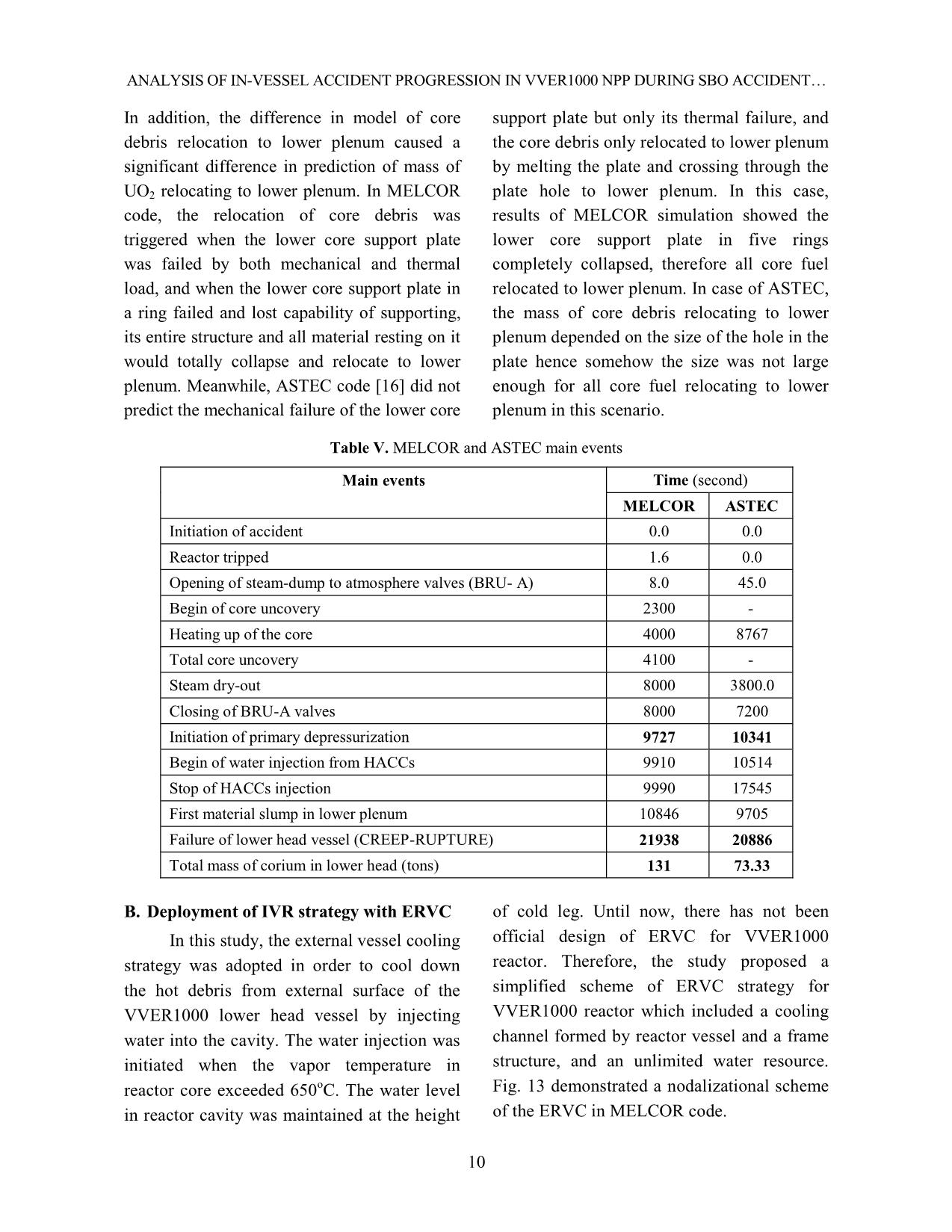
Trang 10
Tải về để xem bản đầy đủ
Tóm tắt nội dung tài liệu: Analysis of in-vessel accident progression in VVER1000 NPP during SBO accident with external reactor vessel cooling method
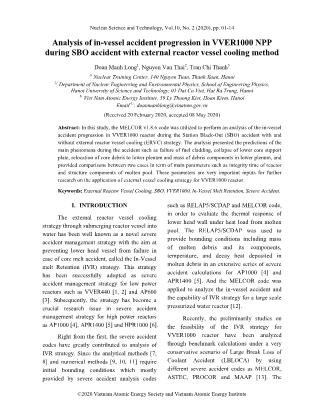
of the segments slightly decreased after the depressurization, they, however, started to DOAN MANH LONG et al. 9 increase right after appearance of core debris in lower plenum. Generally, the first five segments were imposed thermal load more than others which led to higher evolution of their temperatures compared to others. Since 20000 seconds, temperatures of the first five segments were over 1000oK which initiated the thermal strain of the lower head vessel at the segments. The evolutions of thermal strain fraction at 9 segments are displayed in Fig. 12. Among the first five segments, the 3rd segment seemed to be imposed the highest heat hence its temperature was the strongest increase. Due to experience of the highest thermal load, the evolution of elastic strain of the 3rd segment was the sharpest increase and reached to the value of 0.18 at 21938 seconds (6 hours) since initiation of the accident, marked the failure of lower head vessel. Fig. 12. Elastic strain of 9 segments Table IV. Mass of debris in lower plenum Debris Mass (kg) UO2 80600 Zr 18150 ZrO2 8800 Steel 31000 Oxide of steel 2750 Fig. 10 indicates the relocation of core fuels started from 10864 seconds and stopped at 15000 seconds, and together with other components such as ZrO2, Zr and oxides of steel was stable after 15000 seconds. Meanwhile, the evolution of steel debris kept on going due to the collapse of supporting structure in lower plenum and stabilized after 20000 seconds. The results showed all core fuels relocated to lower plenum and according to time relocation of core fuels the decay heat power deposited in debris bed with elimination of decay power of volatile gases varied from 32 MW to 29 MW. At the time of lower head failure, also end of simulation time, total mass of debris components, predicted by MELCOR v1.8.6, are listed in Table IV. A code-to-code comparison for the main events of in-vessel accident progress between MELCOR code in this work and ASTEC in [14] was presented in Table V. The comparison shows the considerable differences of results obtained in both codes. The prediction of ASTEC on relocation of core melt to lower plenum was at 9705 seconds which is 1141 seconds earlier than MELCOR prediction which was at 10846 seconds. As a result, the failure of lower head vessel predicted by ASTEC was at 20886 seconds which is 1052 seconds earlier that MELCOR prediction which was at 21938 seconds. However, MELCOR results predicted a larger mass of corium in lower plenum than ASTEC results which were 131 tons and 73.3 tons respectively. The differences in the time of main events were caused by considerable differences in calculation models in two codes. Besides the reactor trip assumption of ASTEC also contributed to differences in predictions when it was assumed to happen at the same time with initiation of SBO accident which is unrealistic. ANALYSIS OF IN- 10 In addition, the difference in model of core debris relocation to lower plenum caused a significant difference in prediction of mass of UO2 relocating to lower plenum. In MELCOR code, the relocation of core debris was triggered when the lower core support plate was failed by both mechanical and thermal load, and when the lower core support plate in a ring failed and lost capability of supporting, its entire structure and all material resting on it would totally collapse and relocate to lower plenum. Meanwhile, ASTEC code [16] did not predict the mechanical failure of the lower core support plate but only its thermal failure, and the core debris only relocated to lower plenum by melting the plate and crossing through the plate hole to lower plenum. In this case, results of MELCOR simulation showed the lower core support plate in five rings completely collapsed, therefore all core fuel relocated to lower plenum. In case of ASTEC, the mass of core debris relocating to lower plenum depended on the size of the hole in the plate hence somehow the size was not large enough for all core fuel relocating to lower plenum in this scenario. Table V. MELCOR and ASTEC main events Main events Time (second) MELCOR ASTEC Initiation of accident 0.0 0.0 Reactor tripped 1.6 0.0 Opening of steam-dump to atmosphere valves (BRU- A) 8.0 45.0 Begin of core uncovery 2300 - Heating up of the core 4000 8767 Total core uncovery 4100 - Steam dry-out 8000 3800.0 Closing of BRU-A valves 8000 7200 Initiation of primary depressurization 9727 10341 Begin of water injection from HACCs 9910 10514 Stop of HACCs injection 9990 17545 First material slump in lower plenum 10846 9705 Failure of lower head vessel (CREEP-RUPTURE) 21938 20886 Total mass of corium in lower head (tons) 131 73.33 B. Deployment of IVR strategy with ERVC In this study, the external vessel cooling strategy was adopted in order to cool down the hot debris from external surface of the VVER1000 lower head vessel by injecting water into the cavity. The water injection was initiated when the vapor temperature in reactor core exceeded 650oC. The water level in reactor cavity was maintained at the height of cold leg. Until now, there has not been official design of ERVC for VVER1000 reactor. Therefore, the study proposed a simplified scheme of ERVC strategy for VVER1000 reactor which included a cooling channel formed by reactor vessel and a frame structure, and an unlimited water resource. Fig. 13 demonstrated a nodalizational scheme of the ERVC in MELCOR code. DOAN MANH LONG et al. 11 The SBO accident was re-simulated with deployment of ERVC strategy. The results showed the performance of ERVC strategy did not affect the in-core accident progress. The evolution of the accident remained the same as the scenario without implementing ERVC strategy. A comparison of main events between two SBO scenarios with and without deploying IVR strategy is presented in Table VI. The initiation of ERVC strategy was simultaneously happened at 9727 seconds with the primary depressurization when vapor temperature in reactor core exceeded 650oC. Fig. 14 demonstrated water level in reactor cavity and the water level reached the height of cold leg at 12500 seconds, then it was maintained at the level. Figs. 15-17 displayed the temperature of 9 segments of VVER1000 lower head wall in two both scenarios. The solid lines and the dash lines present the results of the scenario without ERVC strategy and with ERVC strategy respectively. The figures indicate the decrease of temperature of lower head walls in both cases happened right after the initiation of depressurization at 9727 seconds. However, in case of implementing ERVC (dash line) the temperature of lower head at 9 segments decreased deeper. Fig. 13. A nodalization for external reactor vessel cooling strategy Fig. 14. Water level in cooling channel and cavity Table VI. Main events of in-vessel accident progression Main events Time (seconds) With IVR Without IVR Initiation of accident 0.0 0.0 Reactor tripped 1.6 1.6 Begin of core uncovery 2300 2300 Total core uncovery 4100 4100 Initiation of primary depressurization 9727 9727 Start of cavity flooding 9727 9727 Begin of water injection from HACCs 9910 9910 Stop of HACCs injection 9990 9990 Start of oxidation 10000 10000 Start of fuel cladding failure 10300 10300 Failure of lower core plate 10846 10846 Failure of lower head vessel (CREEP-RUPTURE) 27341 21938 ANALYSIS OF IN- 12 Fig. 15. Temperature of segments 1-3 Fig. 15 and Fig. 16 indicate the temperature at segments 1-6 started to increase at 15000 seconds, and it strongly increased at 17000 seconds. Meanwhile, Fig. 17 shows the temperature of lower head wall at segments 7-9 was efficiently cooled and kept at low temperature below 600oC. Together with the increase of temperature of segments 1-6, the heat transfer between lower head vessel and water drastically increased as well, which caused the vibration of water level in cooling channel (Fig. 14). Fig. 16 shows the temperature of segments 4-6 was kept under 1000oK (727oC). Fig. 16. Evolution of temperature of segments 4-6 However, Fig. 15 indicates the temperature of segments 1-3 increased beyond 1000oK, among them the temperature of segments 1 and 3 were beyond 1000oK at 24000 and 26000 seconds respectively which caused the occurrence of thermal strain of the segments. Fig. 18 displayed the evolution of thermal strain fraction of all segments. The results show the thermal strain of lower head at segment 1 reached the value of 0.18 at 27341 seconds which marked the failure of VVER1000 lower head reactor vessel. Fig. 17. Evolution of temperature of segments 7-9 Fig. 18. Evolution of thermal strain of segments DOAN MANH LONG et al. 13 IV. CONCLUDING REMARKS In this paper, the in-vessel accident progress during the Station Black-Out (SBO) accident combined with externally vessel cooling was analyzed for VVER1000 reactor by using MELCOR v.1.8.6 code. Some conclusions were given as following: Under SBO accident, only water from four hydro-accumulators could not prevent the collapse of reactor core and relocation of core debris to lower plenum. The collapse of reactor core marked by failure of fuel cladding was occurred at 10300 seconds (2.86 hours), the appearance of first core debris in lower plenum was at 10846 seconds (3.01 hours), and all core fuels relocated to lower plenum at 15000 seconds (4.2 hours). The decay heat power deposited in debris bed locating in lower plenum was estimated from 32 to 29 MW with taking the elimination of decay power of volatile gases into consideration. All the outcomes would be significant information for further study on thermal behavior of debris bed/molten pool in lower plenum and thermal response of VVER1000 lower head vessel as well; In the base case without implementing IVR strategy, the VVER1000 lower head vessel was failed at 21938 seconds (6.09 hours) due to thermal creep-rupture. Meanwhile, in the case of IVR strategy deployment, the results indicated the strategy could not prevent VVER1000 lower head vessel from failure caused by thermal creep- rupture and only prolonged the existence of VVER1000 lower head vessel for 5437 seconds (1.5 hours) compared to the base case; The flat shape of VVER1000 lower head vessel also raises a concern about the occurrence of failure at low position when the critical heat fluxes at the low positions were low as seen in results of ULPU [7]. The results of the study also showed the failure of VVER1000 lower head vessel happened at low positions, therefore, it is necessary to optimize geometry of cooling channel for VVER1000 reactor; In addition, the comparison on the main phenomena during accident progress between MELCOR and ASTEC suggested it is necessary to take further investigation on in- vessel accident progression in case of SBO accident by other severe accident codes in order to set up a range of key parameters for the bounding configuration of molten pool in VVER1000 lower plenum and specify decay heat power deposited molten pool as well. ABBREVIATIONS AMM Accident Management Measures BRU-A A kind of atmospheric dump valves of steam generators CC Cold Collector CL Cold Leg CP Cold Part CV Control Volume ERVC External Reactor Vessel Cooling HACCs Hydro-ACCumulators HC Hot Collector HL Hot Leg HP Hot Part IVR In-Vessel melt Retention LBLOCA Large Break Lost of Coolant Accident LP Lower Plenum MSH Main Steam Header NPP Nuclear Power Plant ANALYSIS OF IN- 14 PORV Pilot-Operated Relief Valve PRZ PRessuriZer SBO Station Black-Out SG Steam Generator SL Steam Line UP Upper Plenum ACKNOWLEDGEMENT The authors are grateful for financial funding of Ministry of Science and Technology and Vietnam Atomic Energy Institute. This work was performed under ministerial project on 05/01/2018. REFERENCES [1]. Tuomisto, H., and Theofanous, T.G. A consistent approach to severe accident management . Nuclear Engineering and Design 148, 171-183, 1994. [2]. Kym l inen, O., et al. In-vessel retention of corium at the Loviisa plant . Nuclear Engineering and Design, 169, 109-130, 1997. [3]. Theofanous, T.G., et al. In-vessel cool-ability and retention of a core melt , DOE/ID-10460, Volume 1, Revised October, 1996. [4]. Esmaili, H., and Khatib-Rahbar, M. Analysis of in-vessel retention and ex-vessel fuel coolant interaction for AP1000 . Energy Research, Inc., ERI/NRC 04-21, NUREG/CR-6849, 2004. [5]. Rempe, J.L., et al. In Vessel Retention strategy for higher power reactors . Final Report, INEEL/EXT-04-02561, 2005. [6]. Ji Xing et al. HPR1000: Advanced pressurized water reactor with Active and Passive safety Engineering 2 79-87, 2016. [7]. Theofanous, T.G., et al. In-vessel cool-ability and retention of a core melt , DOE/ID-10460, Volume 1, Revised October, 1996. [8]. Esmaili, H., et al. An Assessment of Ex- Vessel Steam Explosions in the AP600 Advanced Pressurized Water Reactor , Energy Research, Inc., ERI/NRC 95-211, 1996. [9]. Bui and Dinh. Modeling of heat transfer in heated-generating liquid pools by an effective diffusivity-convectivity approach , In: Proceedings of 2nd European Thermal-Sciences Conference, Rome, Italy, pp. 1365 1372, 1992. [10].Tran, C.T., and Dinh, T.N. The effective convectivity model for simulations of melt pool heat transfer in a light water reactor pressure vessel lower head . Part I&II. Progress in Nuclear Energy 51, 849 871, 2009. [11].Dombrovskii, L.A., et al. Numerical Simulation of the Stratified-Corium Temperature Field and Melting of the Reactor Vessel for a Severe Accident in a Nuclear Power Station . Thermal Engineering, Vol. 45, No. 9, pp. 775-765, 1998. [12]. Yue Jin et al. In-and ex-vessel coupled analysis of IVR-ERVC phenomenon for large scale PWR . Annals of Nuclear Energy 80 322-337, 2015. [13].SANGIORGI Marco et al. In-Vessel Melt Retention Analysis of a VVER1000 NPP . EUR 27951; doi 10.2790/62596, 2016. [14].Polina Tusheva et al. Analysis of severe accidents in VVER1000 reactors using integral code ASTEC . Proceeding of the 17th International Conference on Nuclear Engineering, Brussels, Belgium, 2009. [15].Sandia National Laboratories, MELCOR computer code manuals , Ver.1.8.6, Rev.3 vol. NUREG/CG 6119, 2005. [16].Belon, S., et al. Insight of core degradation simulation in integral codes throughout ASTEC/MELCOR crosswalk comparisons and ASTEC sensitivities studies . The 8th European Review Meeting on Severe Accident Research, Warsaw, Poland, 2017.
File đính kèm:
analysis_of_in_vessel_accident_progression_in_vver1000_npp_d.pdf